Return to:
Encyclopedia Home Page
Table of Contents
Author Index
Subject Index
Search
Dictionary
ESTIR Home Page
ECS Home Page
ENVIRONMENTAL ELECTROCHEMISTRY
Jorge G. Ibanez
Department of Chemistry and Chemical Engineering
Mexican Microscale Chemistry Center
Iberoamericana University
Prol. Reforma 880
01210, Mexico D.F., Mexico
E-mail: jorge.ibanez@uia.mx
(March, 2004)
 |
The search for alternatives to better our environment is imperative. The voluntary or involuntary contributions to its degradation have grown to the point that the health of many people and of ecosystems is seriously threatened. A bird's eye view of the substances involved in the pollution arena allows one to note that most of them can normally be subject to either an oxidation or a reduction. For example, compounds containing highly oxidized chromium are quite toxic whereas their reduced counterparts are much less dangerous (in fact, chromium compounds in reduced form are essential nutrients). In other words, simple electron transfer can change a compounds toxicity, probably as a result of the concomitant geometry and standard potential changes. Likewise, many organic compounds lose their toxicity upon oxidation (often all the way to carbon dioxide). Such an electron transfer can frequently be achieved on an electrified surface (electrode); this opens a wide door for the electrochemical treatment or destruction of pollutants.
In the same vein, the application of an electric signal and the monitoring of the resulting current or voltage can detect most of these substances. A series of electroanalytical techniques offers the possibility of performing a plethora of qualitative or quantitative determinations of pollutants in a variety of matrices. Some examples include: polarography, voltammetry, chronopotentiometry, chronoamperometry, etc. In addition, many substances are analyzed by other techniques that make use of electrochemical detectors. Electrochemical techniques offer increasing degrees of accuracy and detection limits, often involving dramatically lower costs than other techniques.
In addition, electrochemistry offers a series of advantages that in many cases can be used to make "green" processes. Some include the minimization of waste emissions by improved process design involving the minimization of by-product formation, raw material usage, and energy consumption.
In this way, electrochemistry offers important degrees of:
- Environmental compatibility. Electrons are clean reagents per se.
- Energetic efficiency. Electrochemical processes are not subject to some limitations inherent to other processes.
- Cost. Among the reducing agents commonly employed, electrons have the lowest cost per unit of charge. In addition, electrochemical reactors normally do not require moving parts, and thus are mechanically simple and of relatively low maintenance.
- Versatility. The same electrochemical reactor can often be used for a more than one purpose.
- Ease of automation. The main variables in electrochemical reactors are current and voltage that are ideally suited for process automation, optimization, monitoring, and control.
- Friendly requirements. Contrary to other techniques or processes like incineration, supercritical oxidation, wet oxidation, etc., electrochemical techniques normally do not require high temperature or pressure.
- Selectivity. An adequate combination of experimental conditions (electrolyte composition, temperature, degree of convection, applied potential, current) and reactor characteristics (shape, size, construction materials, electrode materials, membranes) can be judiciously selected as to prevent or minimize energy waste and by-product generation. Such by-products not only mean a waste of materials, but also introduce additional difficulties for their separation and/or disposal.
In spite of these advantages, there are also some challenges to be faced:
- Electrode materials may be prone to erosion, complexation, oxidation, wearing, or inactivation.
- Since most of the electrochemical processes are performed in aqueous solutions, solvent decomposition (that is, water oxidation/reduction) is often hard to avoid and thus there is a concomitant energy waste.
- The production of gases from the above decomposition (hydrogen and oxygen) may form explosive mixtures.
- The best electrode materials in terms of durability and inertness frequently involve precious metals, and this increases costs.
- The cost of electricity in many areas is prohibitive.
- Initial capital investment may be large.
- The lack of knowledge or understanding of electrochemistry is perhaps the greatest hindrance for its utilization.
The commercial availability of many electrochemical reactors and systems for the applications discussed below attests to their maturity as competitive technologies in the environmental arena.
The literature on these processes is quite abundant, and this article is not intended as a detailed review. Interested readers are encouraged to seek other general or specific sources according to their needs (see the Bibliography). Instead, a summary of the main concepts as applied to these processes is given below. Note that physical states of reactants or products are only given when they are not aqueous.
Direct and indirect processes
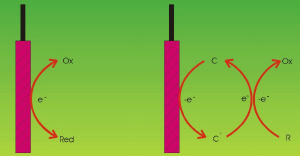 |
Fig. 1. Electron-transfer schemes for direct (left) and indirect (right) processes. |
It is often possible to transfer electrons directly from an electrode to an electroactive species or vice versa. These are called "direct" processes. In other instances this is not possible since the target species may not be electroactive (that is, capable of receiving or donating electrons at an electrode), or else the rate of the reaction may be extremely slow.
To circumvent these impediments one can generate active species (called "mediators") at the electrodes, which are capable of moving into the solution and react there with the target pollutant. Such processes are called "indirect" processes. An advantage here is that the reaction takes place in a homogeneous fashion, and thus the diffusion of the pollutant towards the electrode is not the rate-determining step of the overall process, and thus the effect of low concentrations does not impair the whole reaction pathway. Depending on whether the mediator can be regenerated or not, the process is called "reversible" or "irreversible", respectively. (See Figure 1.)
Direct processes
Direct oxidations
Many organic pollutants (and some inorganics as well) are treated by direct oxidation at anodes of different materials. The materials selection must take into account issues such as cost, accessibility, stability at the required potentials, selectivity, the composition and pH of the reaction medium, the nature of the intermediates and products, and the environmental compatibility. Likewise, the main secondary reaction in aqueous media is water oxidation, which may greatly decrease yields and increase costs (equations of the chemical reactions occurring in this and other processes are shown in the Appendix). In order to avoid the oxidation of water, researchers have developed electrodes that make this reaction to become very slow and thus require an overpotential. A modern example is the boron-doped diamond electrode that is quite durable, resists oxidation, and has a large overpotential for oxygen production; this last property makes possible the oxidation of other substances with standard potentials higher than that for the oxidation of water. Substances that have been treated by this technique include:
Organic compounds.
Phenols, aromatic amines, halogenated compounds, nitrated derivatives, fecal wastes, dyes, aldehydes, carboxylic acid anions, etc.
Inorganic compounds.
Perhaps the inorganic substance that has been most commonly treated by the electrochemical route is cyanide. The main product is the much less toxic cyanate ion.
Direct reductions
In parallel with the above discussion concerning oxidations, the main secondary reaction here is water reduction. The hydrogen thus produced (high purity, electrolytic grade) can sometimes be sold. There are efforts to utilize it in devices such as fuel cells that work with higher efficiencies than the combustion engines.
The electrochemical reduction processes that have reached the highest degree of maturity are undoubtedly those for metal ion recovery from their dissolved ions, as discussed below. Other noxious compounds amenable for direct reduction include:
Organic compounds.
The cathodic dehalogenation of chlorinated hydrocarbons (many of which have been classified as toxic and/or carcinogenic) has a triple advantage:
- electrons are selectively used for the removal of the halide and produce a skeleton amenable for further treatment by cheaper methods (for example, biodegradation),
- chloride ions are produced as by-product, and are not environmentally dangerous, and
- the treatment does not require additional substances to be completed.
Organic acids have also been reduced in this way to the corresponding alcohols or phenols.
Inorganic compounds.
These include chromates, oxychlorinated species (for example, chlorites and chlorates), oxynitrogenated ions (nitrates and nitrites), etc. Metallic ions are treated next.
Metallic ions.
Among the most penalized metals in environmental legislation are: cadmium, copper, chromium, mercury, nickel, silver, lead, and aluminum. Fortunately for our environment, their concentrations are typically small. However this introduces an additional complication for their treatment in electrochemical reactors since mass transport becomes severely limited. To counter this, electrochemists have designed reactors that promote more turbulence and higher contact areas. Three-dimensional and moving electrodes offer promising alternatives, many of which have already been implemented in commercial processes.
Indirect processes
Indirect oxidations
Among the main problems with direct oxidations is the large amount of electrons required for the complete oxidation (that is, all the way to carbon dioxide) as well as the large variety of intermediates. Sometimes the intermediate is even worse than the parent pollutant. Other times, intermediates polymerize and block the electron transfer surface. An alternative to circumvent these problems is to use indirect processes capable of the in situ production of oxidizing or reducing agents at the anode or cathode, respectively. These agents produce oxidation or reduction of pollutants.
Among the most popular oxidants are:
Hydrogen peroxide, which can be formed by the reduction of dissolved oxygen at basic pH.
Metallic ions in higher than normal oxidation states. For example, doubly charged silver cation is an excellent oxidizer for organophosphorous, organosulfur, and chlorinated compounds, both aliphatic and aromatic. Triply charged iron cation is also an oxidizer albeit much weaker that can also be used in successful cases of degradation of grease, cellulose derivatives, urea, meat packing wastes, sewage water, carbonaceous fuels, etc. Cerium ions are well known oxidants used in organic synthesis reactions; they are now used in an environmental application to destroy organic pollutants and munitions residues, converting them into innocuous carbon dioxide. When doubly charged manganese cation is electrochemically oxidized to triply charged cation, these ions can also oxidize pollutants on their own. Insoluble metallic oxides such as bismuth oxide and cobalt oxide have also been proposed for this application.
The production of basic hydroxide ions at the cathode can facilitate removal of water hardness-causing species, for example calcium.
On the other hand it is now common to find wastewaters containing emulsions, formed with water and a dispersed immiscible liquid (for example, in gasoline stations and oil extraction sites). To counter this type of pollution, an electrical field may be used to break up the emulsion since another electrical field can destabilize dispersions formed by charge stabilization. This phenomenon can be coupled to the electrochemical production of flocculating-coagulating agents (like aluminum and iron hydroxides) and the production of a gas (typically hydrogen). As a result of this flocculation-coagulation, pollutants are removed. The resulting solid is a low-density waste due to the flotation action by the gas, which facilitates its separation from the aqueous phase. This is also applied to the removal of dyes that impair the passage of light to the lower parts of aquifers, thus interfering with natural cycles. (Flotation is a process also used in the separation of metal ores.)
Indirect reductions
An example of indirect reductions is that of nitrates and nitrites at electrodes covered with metallophthalocyanines, which act as catalysts. Depending on process conditions, the products may be as innocuous as nitrogen.
Hybrid processes for the treatment of aqueous wastes
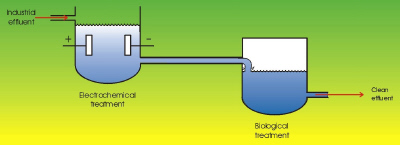 |
Fig. 2. Coupled electrochemical-biological treatments. (From: "Environmental Electrochemistry"
K. Rajeshwar and J. G. Ibanez, Academic Press, 1997.) |
In view of the complexity of the different wastes to be treated by biological processes (some of which are refractory, highly toxic, etc.), some hybrid alternatives offer the possibility for partial electrochemical degradation (for example, the transformation of a non-biodegradable material into biodegradable). This involves large electricity savings, since in order to completely oxidize for example a molecule of benzene, thirty electrons need to be removed! However, partial oxidation of this same molecule can be done to produce the biodegradable organic acids (such as: fumaric, maleic, oxalic, etc.). This process requires much less electrons per molecule. Such products are then amenable for biological treatment. (See Figure 2.) Other alternatives consist of hybridizing electrochemical processes with catalytical, photochemical, sonochemical, or microwave-induced processes.
Processes based on ion exchange
Aqueous industrial wastes can be difficult to treat due in part to their high salinity, high metal content, or pH values that fall outside the limits set by environmental regulations. Then a double incentive becomes attractive since one can prevent pollution, and at the same time also recover valuable materials by recycling, reusing, or recovering metals. An electrochemical method that has been used for quite some time is electrodialysis that is based on the application of an electric field to guide ion migration and through the use of ion-selective membranes concentrate solutions of waste ions and dilute the waste streams. A high degree of selectivity can thus be achieved. Such processes have been successful and their applications extend well beyond the environmental arena. Typical examples include: nitrate removal, boron elimination, desalination of brackish water, nitric acid concentration, glycerin recovery, etc.
Some of the processes involving anion or cation selective membranes are called electrohydrolysis due to the fact that hydrogen ions from water electrolysis hydrolyze the target salt. Some others are called electro-electrodialysis since there is an electrolytic component besides simple electrodialysis. Some examples include:
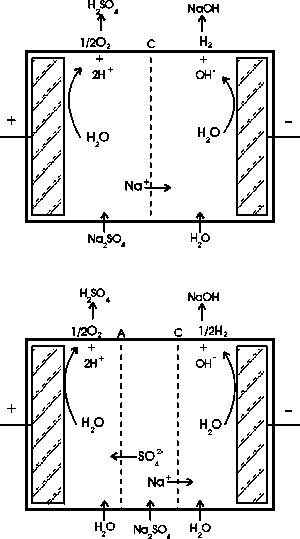 |
Fig. 3. Membrane cell electrolysis; top: two-compartment cell, bottom: three-compartment cell. (From: "Environmental Electrochemistry"
K. Rajeshwar and J. G. Ibanez, Academic Press, 1997.) |
Two-compartment cells.
Here, sodium cations migrate towards the cathode where they encounter the hydroxyl anions produced by water reduction (or else by oxygen reduction). This produces sodium hydroxide. In the same way, hydrogen cations produced at the anode react with the sulfate ions in solution to yield sulfuric acid. In summary, using a waste salt as starting material, the corresponding generating acid ("father" acid) and the generating base ("mother" base) can be recovered. (See Figure 3.)
Three-compartment cells.
Much like the above process, sodium ions migrate towards the cathode and form sodium hydroxide with the hydroxyl ions produced there, while the sulfate ions migrate towards the anode and produce sulfuric acid. The effluent from the central compartment is a dilute solution of the original feed.
Recent developments in the field include the design and production of bipolar membranes, consisting of two membranes "glued" together (one cationic, one anionic); the result is that water is broken at the interface into its component ions: hydrogen and hydroxyl ions that migrate towards the cathode and anode, respectively. During their migration they react with the target salt to yield its "father" acid and its "mother" base. An advantage in using bipolar membranes is the low energy used (since water "dissociates" rather than being "electrolyzed", and the voltage is used for driving the ion migration rather than for electrolysis). The membranes used in these electrochemical processes are typically organic in nature, with ionizable groups bonded to a highly stable (both mechanically and chemically) organic skeleton.
Membrane-based separation technologies are now an important component in many chemical industries and further applications are on sight. In electrochemical applications alone, membrane sales have soared up to 500% in one year.
A clever spin-off technology involves usage of an ion exchange medium (typically resins) bonded onto an electrode surface. The resin adsorbs the target pollutants from an aqueous solution that are later eluted by a polarity change into a separate chamber. This method is called "electrochemical ion exchange (EIX)".
Water disinfection
|
Fig. 4. Electrolytic generation of microorganism deactivators. |
The practice of eliminating unhealthy microorganisms in water dates back to ancient times. There are innumerable treatises on the disinfection of water, including brackish water, sewage water, cooling water, etc. The purpose of this section is to give a brief review of the application of electrochemical methods for the production of disinfection agents.
It is necessary to distinguish here between disinfection and sterilization. The first refers to the deactivation of "pathogen" (disease causing) microorganisms, whereas the second refers to the deactivation of all microorganisms present in the target medium. Mechanisms for such a deactivation include modification of, or attack to:
- The cell wall (rupture, property modification).
- The cell internal components (protoplasm or nucleic acid modification, alteration of protein synthesis, fatal induction of abnormal redox processes).
- The enzymatic activity.
The disinfecting agents most commonly used have properties as oxidants. This makes them useful for the deactivation of most microorganisms, but also brings about undesirable effects such as the discoloration of dyes and the attack of some organic substances. This has an additional drawback in the sense that such effects involve the consumption of extra amounts of the disinfecting agent, thus elevating the corresponding costs. Furthermore, some of these disinfecting agents can produce "disinfection by-products" (DBP) upon their addition or reaction with organic substrates. Such DBP's are frequently toxic, as is the case of most chlorinated hydrocarbons.
The main disinfectants produced by the electrochemical route can be classified according to the oxidizing element as (Figure 4):
- Chlorine-based: chlorine gas, hypochlorite, hypochlorous acid, and chlorine dioxide.
- Oxygen-based: ozone, hydrogen peroxide, and hydroxyl radicals.
- Others: permanganate, ferrate, ions of other transition metal ions (for example copper and silver), percarbonate, persulfate, other halogens and derivatives (for example, bromine, iodine), and the electrochemical production of high levels of acidity or basicity.
Gases
The conventional processes for the remediation of gases are not always flexible enough as to face flow discontinuities, large concentration variations, or the presence of dust particles. On the other hand, wet processes normally consume large amounts of chemicals, and the final products have low commercial value. In addition, sludge generation can be quite large. For these reasons, the electrochemical route has found a market niche for the treatment of various gases, as discussed below. (See the Appendix for some schematic reactions.)
Carbon dioxide
Carbon dioxide reduction is in principle attractive from several stand points. On one hand it is a very abundant substance in the atmosphere, and is a large contributor to the "green house effect". If a synthetic route based on carbon dioxide could be found, not only humanity would have an almost inexhaustible source of raw material, but also the danger of global warming could be greatly reduced. Carbon dioxide is relatively easy to handle, and has very low toxicity. Its reduction products are quite varied according to the reaction conditions, ranging from saturated hydrocarbons (with four or less carbon atoms) and ethylene, to small linear alcohols (with three or less carbon atoms), carbon monoxide, formic acid, and oxalic acid. However, it is not clear at present whether these reactions can ever be made energetically and economically viable.
Nitrogen oxides
Nitric oxide is the most problematic oxide of this family due to its low solubility in water that impedes its removal from a gas stream by absorption in aqueous solutions. This is the main component of chimney gases. Reactive dissolution can do the trick by oxidizing it, or by forming a soluble nitric oxide complex (for example with iron aminopolycarboxylic chelates). It can then be reduced at a later stage.
Nitrogen dioxide is soluble and can be electrochemically reduced by direct or indirect processes. For example, direct reduction in an acid solution can yield nitrogen gas; an indirect method with thiosulfate (that can then be regenerated) also produces nitrogen.
Nitrous oxide is also much more soluble than nitric oxide and can be captured by solutions of amines; it can also be electrocatalytically reduced to nitrogen in basic or acidic media.
Sulfur-containing gases
The sulfur geochemical cycle is among those that has been most altered by human activity, mainly fossil fuel and biomass combustion. Most sulfur in the gas phase is in the form of sulfur dioxide (and a smaller portion as hydrogen sulfide).
Hydrogen sulfide.
Once removed by absorption, this gas can be recovered by the thermal regeneration of the absorbing liquid and sent to the Claus process for the recovery of elemental sulfur (See the Appendix).
Unfortunately this process has several limitations, as the hydrogen from hydrogen sulfide is essentially wasted (since it forms water); also, the required conditions are not flexible enough as to withstand sensible variations in hydrogen sulfide composition, and post-treatments are required. Some modern methods involve the use of oxidants such as hydrogen peroxide, and highly oxidized iron species that can often be regenerated in an external cell process and returned to the absorber unit.
Sulfur dioxide.
"Flue Gas Desulfurization" processes (FGD) remove sulfur dioxide by its irreversible reactive absorption with lime or limestone, or by its reversible absorption in sodium citrate solutions. There are many electrochemical methods that have been studied for this purpose. Most of these involve the direct or indirect oxidation of sulfur dioxide to sulfate ion, although some involve its reduction to elemental sulfur.
Sulfur trioxide.
A technically and economically viable process for the selective removal of sulfur trioxide from thermoelectrical plants uses high temperature, 500oC (932oF), for its selective removal by a molten electrolyte.
Other gases
Hydrocarbons.
Solid electrolyte cells provide heterogeneous catalysis for their treatment by oxidation.
Chlorine.
Waste and impure chlorine can be treated by its dissolution in a metal ion solution, whereby chlorine oxidizes metallic ions and becomes reduced to chloride ions. These are then sent to the anodic compartment of a divided cell to be re-oxidized and produce pure gaseous chlorine.
Electrokinetic treatment of soils
|
Fig. 5. Electrokinetic remediation of soil. (From: "Environmental Electrochemistry"
K. Rajeshwar and J. G. Ibanez, Academic Press, 1997.) |
The pollution of soils, sediments and wetlands is a very serious problem. The application of electric fields has gained considerable ground for the treatment of a wide variety of polluted soils. It is based upon the passage of electrical current through electrodes strategically buried underground. This originates the movement of charged (and some uncharged) species due to three main phenomena: electrophoresis, electroosmosis, and electromigration. (Figure 5.)
Electrophoresis involves the movement of charged particles (typically colloids) under the influence of an electric field.
Electroosmosis involves the movement of the solvent (typically water) within the soil pores, due to the formation of an electric double layer between charged surface particles and dissolved ions or solvent dipoles. The external field then attracts the solvent, which in turn drags dissolved species. This phenomenon is known since the middle of the past century by civil engineers, who use it for the removal of humidity from soils, walls, and roofs. Waste wet solids or sludge can also be dewatered in this way.
Electromigration consists of the movement of ionic species in the liquid phase towards the oppositely charged electrode.
Electrokinetic remediation (also called electroreclamation, electrorestoration, electroremediation, electrokinetic processing, or electropotential ion transport) has been used for a variety of applications. Among the species in soils that have been treated with this technique are organic substances (benzene, toluene, ethyl benzene, xylenes, gasoline, warfare agents, acetates, phenols, trichloroethylene, etc.), inorganic species (metallic ions of zinc, mercury, cadmium, nickel, lead, chromium, copper, iron, and silver, in addition to anionic forms of arsenic, chloride, nitrate, sulfate, and cyanide anions, complexes, etc.) as well as radioactive substances. This technique is also in use for the clean up of sludge and groundwater.
Electrokinetic remediation is also used in combination with biological remediation in cases where the organic pollutant is very insoluble or else has a large mass/charge ratio. The problem with soil bioremediation alone is the difficulty for the transportation of oxygen and nutrients to microorganisms, and the requirement of temperatures somewhat higher than ambient. Fortunately, electrokinetic remediation is capable of transporting the necessary oxygen and nutrients in the remediation fluid; also, the passage of electrical current generates some heat that helps to reach the necessary temperature for bioremediation, and facilitates the movement of microorganisms in a directed way (after all, they have a certain surface charge). Thus, the combination of both techniques is a promising route. Numerical simulations of electrokinetic phenomena are an important aid in the calculation of the required parameters for an efficient use of the current.
Electrochemical recycling
The recycling of pollutants is one of the main ways in which an industry can balance its productive activity with environmental care. If a process consumes recyclable materials it is advantageous from at least two standpoints, since it does not add to pollution and it recovers useful materials. In this way, electrochemical processes can be used for the recycling of toxic materials used as reagents or catalysts in chemical processes. For example, a highly oxidized chromium species is used as an oxidizer in the synthesis of anthraquinone. The resulting reduced chromium species can then be electrochemically reoxidized, and reused in the main process. A field in which this approach is quite promising and that has not been fully exploited is that of battery and catalyst recycling.
Interestingly, oxidized electronic components that cannot be soldered due to the presence of oxide layers on their surfaces can be chemically reduced to restore their solderability, and the spent reducer can be electrochemically regenerated.
Commercial applications
Many of the techniques discussed in this review have reached the commercial stage. This means that they are competitive with other alternative techniques. In this brief review they cannot be summarized. For example, effluents from the following industries or activities are electrochemically treated: pharmaceutical, textile, veterinary, food processing, polymers, chemicals, pulp and paper, agricultural, etc. As discussed above, many soils, gases, and hazardous, toxic, or radioactive wastes are now treated electrochemically. The book written by Rajeshwar and Ibanez gives an account of companies devoted to these endeavors (see the Bibliography).
Concluding remarks
Electrochemical techniques and processes offer important alternatives for the monitoring, prevention, and remediation of the environment. This overview focused on the remediation aspect, emphasizing direct and indirect processes, with discussions of applications for the treatment of gases, liquids, and soils as well as for water disinfection. The battle to preserve our environment is a fierce one; hopefully this account gives an overview of many of the ways in which electrochemistry contributes with its best munitions.
Appendix
Some reactions occurring in the above described processes
[1] |
2H2O(l) ==> O2(g) + 4H+ + 4e- |
(water oxidation) |
[2] |
2H2O(l) + 2e- ==> H2(g) + 2OH- |
(water reduction) |
[3] |
RCl + 2H+ + 2e- ==> RH + HCl |
(dehalogenation of hydrocarbons) |
[4] |
HCO3- + OH- ==> CO32- + H2O(l) |
(hardness removal 1) |
[5] |
Ca2+ + CO32- ==> CaCO3(s) |
(hardness removal 2) |
[6] |
H2S(g) + 3/2O2(g) ==> SO2(g) + H2O(g) |
(Claus process 1) |
[7] |
H2S(g) + SO2(g) ==> 2S(g,l) + H2O(g,l) + 1/2 O2(g) |
(Claus process 2) |
Electrochemical treatment of gaseous pollutants
The only products shown are those that contain the main atom. Reactions are not balanced, and physical states are omitted for clarity.
CO2 + (ne- + nH+) ==> (HCOO)2, HCOOH, CO, C, HCHO, CH3OH, CH4
H2 ==> S, S2, SO42-, H2S(conc) + (ne- + H2)
SO2 ==> SO3, H2SO4 + (ne-)
SO2 + (ne-) ==> S
NO + (ne- + nH+) ==> N2, N2O, NH2OH, NH3
NO2 + (ne- + nH+) ==> NH3
N2O + (ne-) ==> N2
Bibliography
- Environmental Electrochemistry,
K. Rajeshwar and J. G. Ibanez, Academic Press, San Diego, CA, 1997.
- Electrochemical Membrane Flue-Gas Desulfurization: K2SO4/V2O5 Electrolyte,
D. S. Schmidt and J. Winnick, "American Institute of Chemical Engineers Journal" Vol. 44, pp 323-331, 1998.
- Steady State and Limiting Current in Electroremediation of Soil,
J. M. Dzenitis, "Journal of The Electrochemical Society" Vol. 144, pp 1317-1322, 1997.
- Ferrate(VI) Oxidation of Hydrogen Sulfide,
V. K. Sharma, J. O. Smith, and F. J. Millero, "Environmental Science and Technology" Vol. 31, pp 2486-2491, 1997.
- Environmental Oriented Electrochemistry,
C. A. C. Sequeira (editor), Elsevier, Amsterdam, 1994.
- Electrochemistry for a Cleaner Environment,
D. Genders and N. Weinberg (editors), The Electrosynthesis Company Inc., East Amherst, NY, 1992.
- Industrial Electrochemistry (2nd edition),
D. Pletcher and F. Walsh, Chapman and Hall, London, 1990.
Listings of electrochemistry books, review chapters, proceedings volumes, and full text of some historical publications are also available in the Electrochemistry Science and Technology Information Resource (ESTIR). (http://knowledge.electrochem.org/estir/)
Return to:
Top
Encyclopedia Home Page
Table of Contents
Author Index
Subject Index
Search
Dictionary
ESTIR Home Page
ECS Home Page
|