Return to:
Encyclopedia Home Page
Table of Contents
Author Index
Subject Index
Search
Dictionary
ESTIR Home Page
ECS Home Page
ELECTROLYTIC CAPACITORS
Sam Parler
Cornell Dubilier Electronics, Inc.
140 Technology Place
Liberty, SC 29657, USA
E-mail: sparler@cde.com
(March, 2005)
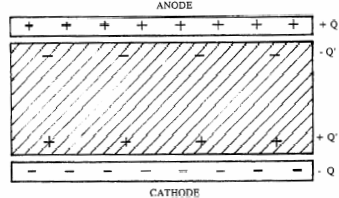 |
Fig. 1. Charges "Q" on anode and cathode induce charges "Q'" on the dielectric. |
Capacitors store energy as well as charge. These charges are generally stored on conductive plates, the positively charged plate called the anode and the negatively charged plate called the cathode (Figure 1). In order to keep the charges separate, the medium between the anode and cathode, called the dielectric, must be non-conductive an electrical insulator. The anode and cathode are configured so that very little movement occurs between them as they are charged and the force on the dielectric increases. As the stored charge increases, the electric field across the dielectric increases. This situation gives rise to a voltage which increases proportionally with the charge. The ratio of the charge magnitude on each plate to the electric potential (voltage) between the plates is known as capacitance. The energy stored in a capacitor is the energy required to move the stored charge through the potential of the capacitor. The capacitance of a device depends mostly on the plate geometry and the nature of the dielectric. It is directly proportional to the "dielectric constant" (Table I) and inversely proportional to the thickness of the dielectric. See the Appendix for more details.
|
Table I. Relative dielectric constants "k" for various dielectrics |
| | |
Dielectric material | Relative dielectric constant "k" |
| | |
Air or vacuum | 1.0 |
Polypropylene | 2.2 |
Polyester (Mylar) | 3.0 |
Mica | 6.0 |
Aluminum oxide | 8.5 |
Tantalum pentoxide | 27 |
Niobium oxide | 41 |
Barium titanate | 1,000 - 10,000 |
|
As the charge and voltage on a given capacitor are increased, at some point the dielectric will no longer be able to insulate the charges from each other. The dielectric then exhibits dielectric breakdown, or high conductivity in some areas, which tends to lower the stored energy and charge, generating internal heat. This phenomenon, undesirable in most capacitor applications, occurs at the capacitor's breakdown voltage. Capacitor damage or destruction can occur in such situations. Normally, breakdown ratings for dielectrics are expressed as a maximum field strength which is basically the ratio of the applied voltage to the dielectric thickness.
The mass energy density of a capacitor is the ratio of the amount of energy the capacitor can store at its working voltage to the capacitor mass, including package. The working voltage of a capacitor is defined as the maximum rated voltage for a given application. The working voltage is generally less than the breakdown voltage. An exception to this rule could occur if the transient peak voltage were able to exceed the steady-state breakdown voltage. The volumetric energy density of a capacitor is defined as the ratio of the energy stored to the capacitor volume, including package.
The first capacitor was invented in 1745 by Pieter van Musschenbroek, a physicist and mathematician in Leiden, Netherlands (and called the Leyden jar). It was a simple glass jar coated inside and outside with metal foil. William Dubilier invented the mica capacitor in about 1910.
Electrolytic capacitors
Electrolytic capacitors are capacitors in which one or both of the "plates" is a non-metallic conductive substance, an electrolyte. Electrolytes have lower conductivity than metals, so are only used in capacitors when metallic plate is not practical, such as when the dielectric surface is fragile or rough in shape or when ionic current is required to maintain the dielectric integrity. The dielectric material of electrolytic capacitors is produced from the anode metal itself in what is known as the forming (or anodizing process. During this process, current flows from the anode metal which must be a valve metal such as aluminum, niobium, tantalum, titanium, or silicon through a conductive bath of a special forming electrolyte to the bath cathode. The flow of current causes an insulating metal oxide to grow out of and into the surface of the anode. The thickness, structure and composition of this insulating layer determine its dielectric strength. The applied potential between the anode metal and the bath cathode must be above the oxide breakdown voltage before significant current will flow. As current flows, the breakdown strength (formed voltage) and oxide thickness increase. See Figure 2 for a comparison of the electrostatic (classical) and electrolytic capacitors. The "electrolytic capacitors" are quite different from, and not to be confused with the "electrochemical capacitors" (also called ultracapacitors) the operation of which is based on the electrical double layer capacitance.
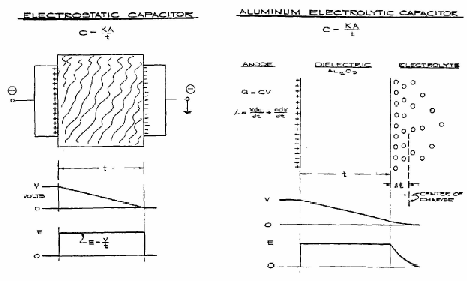 |
Fig. 2. Comparison of electrostatic and electrolytic capacitors. |
The electrolysis reaction was investigated by Michael Faraday in the 1700's. A relationship between the charge flow through the system and the amount of product (in this case, metallic oxide) was found to exist. Faraday noted the relationship between gram-equivalents of product and charge transfer for all ideal (stoichiometric) electrolysis reactions in what is now known as Faraday's Law. Departures from this relationship exist for the process of oxide formation on anode metals, since some oxide can be grown chemically and thermally to reduce the electrical energy requirements in the formation process, which can cost several dollars per kilogram of anode produced. Also there can be some undesirable side reactions during the formation process which do not contribute to oxide formation. In the formation process, brittle metallic oxide is grown upon the metal foil, which is usually rough in shape. The anode metal is therefore in intimate contact with one side of the oxide dielectric. The electrolyte is used to make contact between the other side of the oxide and the cathode plate.
The advantage of electrolytic capacitors is the high capacitance per unit volume and per unit cost. The high capacitance arises from the high dielectric constant, the high breakdown field strength, the rough surface, and the extremely small, uniform thickness of the anodically formed metallic oxide. The reason that electrolytic capacitors have such uniform dielectric stress and can operate at such high field strength, within 80% of their breakdown strength, on the order of 1,000 volts/µm, is due to two reasons. First, the original anodization ("formation") process is performed at a fixed voltage, and the dielectric grows everywhere to whatever thickness is required to support that voltage. Second, once the foil is in a capacitor, the capacitor "fill" electrolyte continues the healing work of the original forming electrolyte, repairing and thickening the dielectric locally as required. This healing process is driven by the capacitor's dc leakage current, which is drawn whenever a dc voltage is applied to the capacitor, that is, whenever it is in operation. In fact, electrolytic capacitors often last longer when they are in continuous, mild use that when they are only charged up briefly every year or decade.
The disadvantage of electrolytic capacitors is the non-ideal, lossy characteristics which arise from the semiconductive oxide properties, double-layer effects from the electrolyte-oxide charge-space region, resistive losses from the high electrolyte resistivity, frequency response rolloff due to the roughness of the surface oxide, and finite capacitor life due to breakdown and degradation of the electrolyte. Some of these considerations will be discussed below in more detail from the standpoint of the aluminum electrolytic capacitor.
Also, the anodic oxide dielectric is polar, and so are the electrolytic capacitors (in contrast with the classical, electrostatic capacitors), that is the capacitors must be connected with the correct polarity as marked. Connecting with reverse voltage injects hydrogen ions through the oxide readily, causing high electrical conduction, heating and reduction of the anodic oxide film. Non-polar (or bi-polar) devices can be made by using two anodes instead of an anode and a cathode, or one could connect the positives or negatives of two identical device together, then the other two terminals would form a non-polar device.
Most electrolytic capacitors are constructed using aluminum electrodes, but tantalum and niobium is also used. Aluminum anode is the least expensive at $0.04 per gram. As such, it is used in large (even greater than one liter!) and small (tiny surface-mount) capacitors. Tantalum anode material is over $2.00 per gram but offers high stability, more capacitance (four times that of aluminum), lower resistance (up to 90% lower) per size. It is available as small units (typically less than 5 cm3) and surface mount. Niobium anodic powder is less than $1.00 per gram, much cheaper and more available than tantalum but still much more expensive than aluminum. Capacitance is much more than aluminum, nearly that of tantalum. It is a much newer technology than tantalum.
H.O. Siegmund invented the electrolytic capacitor in 1921. Julius Lilienfeld did much to develop electrolytic theory in the 1920's and 1930's. Cornell Dubilier was at this time the world's largest capacitor company, and did much to develop the technology of etching and anodizing.
Construction details of a wet aluminum electrolytic capacitor
Production process
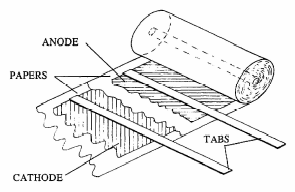 |
Fig. 3. Construction of an electrolytic capacitor. |
Aluminum electrolytic capacitors are comprised of anode and cathode plates separated by an absorbent spacer. As shown in Figure 3, metal tabs are attached to the anode and cathode plates, and the assembly is wound into a cylindrical section. The tabs are welded to aluminum terminals installed in a header (top). The section-header assembly is immersed in a bath of hot capacitor electrolyte (significantly different from the formation process electrolyte). In what is called the impregnation process, a vacuum is applied to the electrolyte and sections, causing electrolyte to be drawn into the sections, thoroughly wetting the sections. The sections are placed in aluminum cans, and the headers are sealed to the cans. The capacitor units are slowly brought up to maximum rated voltage at maximum rated temperature during the aging process. The aging process grows oxide on areas on the anode foil which have an insufficient oxide barrier, such as slit edges and places which have been cracked during the winding operation. Inspections and tests occur at several stages of the production process.
Anode
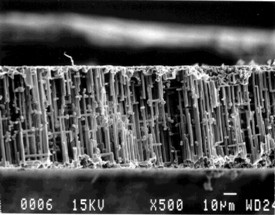 |
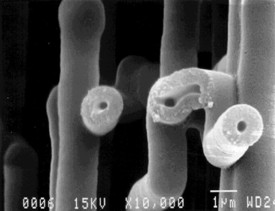 |
Fig. 4. Scanning electron microscope edge view of a sheet of the oxide tunnels after the surrounding aluminum was dissolved (top), and a closer view of some oxide tunnels (bottom). |
The anode can be made of various purities of aluminum, but for high voltage, high energy density aluminum electrolytic capacitors, the anode is generally comprised of 99.99% purity, high cubicity aluminum foil of about 100-micrometer thickness. The term high cubicity refers to the rectangularly oriented aluminum grain structure which is intentionally produced in the foil. The anode foil is generally produced in rolls of 270-kg mass and 48-cm width. The first production process this foil undergoes is called etching, which electrochemically roughens the surface of the foil, causing hollow tunnels to grow into the aluminum. The rectangular aluminum grain orientation causes the etched tunnels to form along parallel paths which are mostly perpendicular to the aluminum top surface. The etching process greatly increases the microscopic to macroscopic surface area ratio, called the "foil gain", which can be up to sixty for high-voltage aluminum electrolytic anode foil, and even higher for low-voltage foil. The foil emerges from the etching process considerably lighter in weight than it entered. The next process the roll of foil undergoes is called the formation process. Aluminum oxide is grown onto and into the hollow tunnels which were etched into the aluminum during the etching process. Figure 4 shows the scanning electron microscope edge view of a sheet of the oxide tunnels after the surrounding aluminum was dissolved and a closer view of some oxide tunnels. Notice that the inside diameter of this 550-volt oxide tunnel is about a quarter of a micrometer and that the wall thickness is a little more than one-half micrometer. In general, the ratio of the oxide thickness to the oxide formation voltage is about 1.0 nanometer per volt. This ratio varies somewhat with the oxide structure. Depending on the formation electrolyte composition, the current density, and other production parameters, the structure of the aluminum oxide may be amorphous, crystalline, hydrous, or some combination of these structures
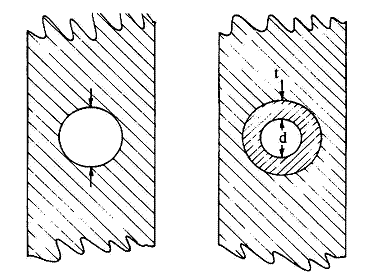 |
Fig. 5. Anode tunnel cross-section before (left) and after (right) the oxide formation process. |
The etching and forming processes must be compatible to achieve good results. Figure 5 shows a cross-section of a tunnel in the anode aluminum before and after forming. Note that the relationship between etched tunnel diameter and the formation voltage (thickness) is important. Since the oxide grows inward as well as outward in the tunnel, if the etch diameter is too small, the tunnel can clog or completely fill with aluminum oxide during the formation process, thus contributing little to the foil capacitance, since the electrolyte cannot contact the inside of the tunnel. If the tunnel diameter is too large, the optimum capacitance cannot be realized due to wasted space. The combination of the etching and forming processes determine the formation voltage "Vf" and the gain of the foil produced. The gain is defined as the capacitance per unit macroscopic area of the produced foil divided by the capacitance per unit area of unetched foil of the same formation voltage. High energy density aluminum electrolytic capacitors use high-gain foil. It may be noted here that the breakdown field strength of 1 V per 1.0 nm is much higher than for polymer films. The "k" value of 8.5 for aluminum oxide is also about three times larger than for most film dielectrics (see Table I). Aluminum oxide is several times denser than polymeric films, however.
Cathode
The cathode aluminum foil is generally thinner than the anode and must exhibit much higher capacitance than the anode, since the cathode capacitance appears in series with the anode capacitance to yield the total capacitance (See the Appendix). For a given anode capacitance, the maximum total capacitance occurs when the cathode capacitance is as large as possible. High cathode capacitance requires a very low cathode formation voltage. Generally the cathode is not formed at all, but there is always a thin layer (around 2-3 nm) of hydrous oxide on the surface of aluminum unless it is passivated and the electrical double layer also has a large capacitance. A thin hydrous oxide layer forms readily on aluminum with exposure to normal air in the atmosphere. Titanium passivation of cathode foil has been undertaken in recent years to offer a cathode with a capacitance approaching 200 µF/cm2. Such a high cathode capacitance is necessary only for low-voltage capacitors with high-gain anodes. Generally a cathode capacitance of fifty times the anode capacitance is sufficient. This situation yields a total capacitance which is only 2% less than the anode capacitance. For a discharge capacitor, the charge on the anode plate must be neutralized by the opposite charge on the cathode plate, which requires that the cathode be capable of storing a charge greater than or equal to the anode charge. In other words, the product of the capacitance and forming voltage must be larger for the cathode than for the anode. This requirement is generally met automatically, since the charge storage capability of formed foil is maximized at low formation voltage. A thin foil with a surface etch is used for the cathode, giving a frequency response generally better than that of the anode, and giving a large enough capacitance that the total unit capacitance is not diminished. Since the cathode's voltage capability is usually only about one volt, the electrolytic capacitor unit is limited in its steady-state reverse voltage capability to about one volt. It has been found that in some cases transient reverse voltages in excess of 100 volts may appear on the capacitor for durations of around one millisecond with no detrimental effects for thousands of cycles; however it is not clear what the actual cathode potential is in these cases. What is known is that extended reverse voltage for time intervals as short as one second can cause significant heating of the electrolyte and of the anode oxide. The current drawn during these reverse voltages can easily reach hundreds of dc amperes. Electrolytic capacitors can be constructed with formed cathodes to allow voltage reversal without damage. The drawbacks to such construction are reduced total capacitance, since the anode and cathode are in series; and reduced energy density, due to the decreased capacitance and increased mass from the heavier, formed cathode.
Separator
The separator or spacer is an absorbent material in roll form which is wound between the anode and cathode to prevent the foils from coming in contact with one another. The spacer is generally made of paper, which can be of many different types, densities, and thicknesses, depending on the voltage and effective series resistance requirements. Besides separating the anode and cathode, the spacer must wick and hold electrolyte between the plates. The resistance of the spacer-electrolyte combination is appreciably greater than would be accounted for by its geometry and the resistivity of the absorbed electrolyte. The electrolyte-spacer combination also impacts the capacitor's
frequency response.
Electrolyte
The primary purpose of the electrolyte is to serve as a "plate" on the outer anode oxide surface and also to connect to the cathode plate. The electrolyte is a high-resistivity, high-dielectric-constant, high dielectric-strength organic liquid solvent with one or more dissolved, ionically conductive solutes. The secondary purpose of the electrolyte is to repair, heal, or insulate defect sites in the anode aluminum oxide during the application of voltage between the anode and cathode.
Tabs
Tabs are strips of aluminum which make contact between the conductive plates and the connection terminals in the header. There may be several tabs connected to each of the plates. Each tab is either cold-welded or staked along the entire width of the anode and cathode foils. The tab paths are generally run from the capacitor section to the terminals in a fashion which keeps the inductance low and prevents tabs of the opposite polarity from coming in contact with one another or the case during movement and vibration of the capacitor unit. The tabs are spot-welded to the underside of the terminals in the header assembly. Tab material is not etched, but is formed to a high voltage prior to its assembly into a capacitor. Optimum tab placement along the foil is considered to be the placement that minimizes power loss due to the metal foil resistance. This optimum leads to equal spacing from each tab to the one nearest it, and half of the inter-tab distance is provided between the outermost tabs and the foil ends. For high voltage capacitors, the tab resistance and metal foil resistance are quite small compared to the oxide and electrolyte resistance.
Package
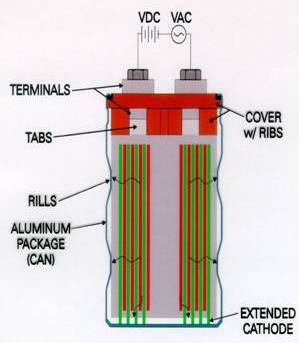 |
Fig. 6. Schematic of the capacitor package. |
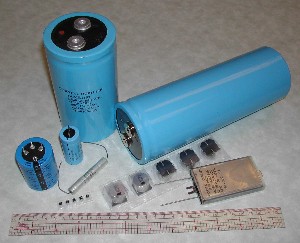 |
Fig. 7. Some electrolytic capacitors. |
The can into which the capacitor section is placed is made of 1100-alloy aluminum, which is of around 99% purity (see Figure 6). For 25 to 50 mm (one-inch to two-inch)-diameter capacitors, the wall thickness is 0.022 inches. A butyl rubber gasket is placed on top of the header before the spinning operation, in which the case opening is folded over and pressed into the gasket, forming an effective seal of the system. The package is at the same potential as the electrolyte and cathode during capacitor operation, so when electrolytic capacitors are connected in series, care must be taken to insulate the cases from one another. Although the aluminum electrolytic capacitor case is at the cathode potential, it may not be used for the negative electrical connection because of high electrolyte resistivity and the long effective path from the cathode to the can. If the electrolyte were of much lower resistivity, eliminating the cathode and using the can instead might be a possibility. A safety vent is provided in capacitors so that the capacitor may relieve excessive pressure buildup in a controlled manner. This occurrence is known as venting, and is considered a failure mode. The vent may be installed as a rubber plug in the header or as a die-set slit impression in the can wall. The pressure at which the capacitor vents is predictable, and is usually designed to occur at about seven atmosphere pressure or even higher. The allowable pressure tends to be higher for small capacitors. After a capacitor vents, the electrolyte may evaporate out until the capacitance diminishes. Some typical electrolytic capacitors are shown in Figure7.
Uses and applications of electrolytic capacitors
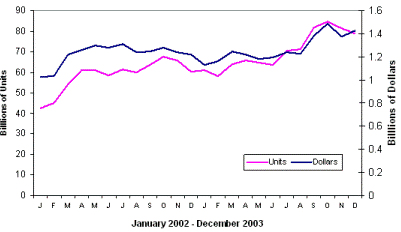 |
Fig. 8. Monthly global capacitor market. |
There are many practical, everyday applications of aluminum electrolytic capacitors. The most important applications include filter capacitors for power supply outputs, blocking and dc-bypass circuits, motor start and other non-polarized capacitors, audio applications, energy discharge capacitors, photoflash and strobe capacitors. Each of these require quite different characteristics and those are detailed in the Appendix.
Total worldwide usage of capacitors is approximately one trillion units per year. The total market value is approximately 17 billion dollars per year. Figure 8 show the monthly fluctuations of the total capacitor market for a couple of recent years. And Figure 9 presents the annual markets for aluminum and tantalum electrolytic capacitors, which are more than 10% of total usage.
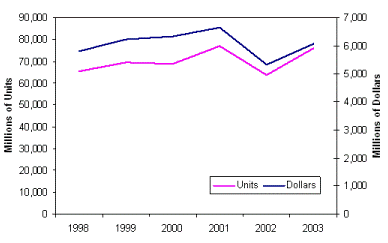 |
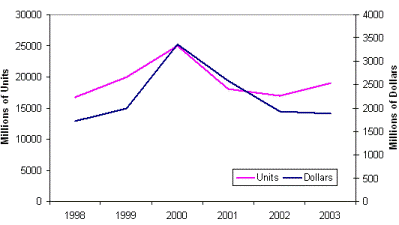 |
Fig. 9. Global electrolytic capacitor market: aluminum (left), tantalum (right). |
Appendix
The ratio of the charge magnitude "Q" on each plate to the electric potential or voltage "V" between the plates is known as capacitance "C".
[1] 
The capacitance of a device depends mostly on the plate geometry and the nature of the dielectric. For two parallel surfaces each having area "A" separated by distance "d" with a dielectric of relative dielectric constant "k":
[2] 
where "Eo" is the dielectric constant of vacuum (8.85×10-12 F/meter). The relative dielectric constant "k" of a material describes its polarizability. As seen in Figure 1, as charges +Q and -Q are established on the anode and cathode plates, respectively, surface charges of +Q' and -Q' on the dielectric are induced according to the following relation which defines "k" for the material: Q' =Q×(1-k).
The cathode capacitance "Cc" is in series with the anode capacitance "Ca" to yield the total capacitance "C" according to the relation:
[3] 
Or, rearranging:
[4] 
Consequently, in series coupled capacitors the lower value capacitor dominates.
Uses and applications of electrolytic capacitor
Power supply output filter
When a sinusoidal ac voltage is rectified, a semi-sinusoidal waveform is produced. This waveform is generally converted to a steady dc value by use of a capacitor, which charges to the peak value of the semi-sinusoidal voltage, then provides current to the load at a slightly decreasing voltage until the next half-sine peak restores the maximum voltage to the capacitor. The slight variation in the capacitor voltage is known as the ripple voltage and the current going to and from the capacitor is called the ripple current. To maintain a steady dc output and minimize the ripple voltage, the capacitor's capacitance is selected sufficiently large with respect to the load resistance. A steadier voltage requires a higher value of capacitance and a more costly capacitor. For applications in which the steadiness of the voltage is not very critical, a smaller capacitance is often selected. The ripple current must then be taken into account, for too small a capacitance may have a large effective series resistance (ESR) and may tend to overheat. Maximum ripple current ratings are specified by capacitor manufacturers, and these ratings are derived from the maximum allowable operating temperature of the capacitor as well as the size, mass, construction materials, and ESR of the capacitor. Ripple current ratings in aluminum electrolytic capacitors are available as high as 50 amps rms.
Blocking and dc bypass
The frequency response characteristic of a capacitor is such that it appears to be an open circuit to a steady, dc voltage and a virtual short circuit to high frequencies. As such, the capacitor can be used to route signals according to their frequency content. When a signal containing both dc and ac components is sent to a transformer to amplify the ac portion, often a capacitor is used in series with the transformer to block the dc component, which would cause heating and signal distortion were it to reach the transformer. For such an application the linearity of the frequency response of the capacitor needs to be examined to ensure high fidelity, and the magnitude of the capacitor current needs to be lower than its rated ripple current.
Motor start and other non-polar
The starting torque of ac motors is provided by a motor-start capacitor, often a low-ESR bi-polar aluminum electrolytic capacitor. Such a capacitor is designed for line ac voltage, high current, short-duty operation. Motor-start capacitors have the lowest dissipation factors of the aluminum electrolytics, as low as 2% at 120 Hz. Low-gain foil is used in order to achieve such low ESR. The cases are often made of plastic to provide electrical isolation from the electrolyte potential, which follows the applied voltage. The energy densities are quite low, generally 50 J/kg or less. Even with these low-loss properties, motor-start capacitors quickly heat up in their application, and are only recommended for low duty cycles, such as one second on, one minute off.
Audio applications
Non-polar aluminum electrolytics of 50 and 100-volt ratings are often used in passive crossovers for commercial and consumer loudspeakers, where the signals contain medium ac voltage components (around 30 volts peak) with little or no dc voltage content. Frequency response and vibration resistance of these capacitors are the most important criteria. Electrolytic capacitors have a positive voltage coefficient of capacitance, and this leads to some harmonic distortion.
Car audio (bus stiffening): One ideal application is for large, multi-kilowatt car audio amplifier bus stiffening applications, where the 13 V dc rail may have hundreds of amps peak drawn with every bass drum kick or every bass guitar slap or pop. This can cause the car battery voltage to drop by several volts, dimming the headlights in rhythm to the music and lessening the life of the alternator and battery, not to mention to degrading the audio distortion and output levels. The solution is to use electrolytic capacitors near the amplifiers. These special-purpose capacitors have ratings from 0.5 to 2.0 farads at 15 V dc. These capacitors usually have a series resistance near one milliohm, so are quite effective at stiffening the car's battery voltage, when used at a level of about 1 farad per kilowatt. Future capacitors will probably be rated 0.2-0.5 F at 60 V dc for the higher battery voltages.
Energy discharge applications
The usual energy discharge application for aluminum electrolytic capacitors is in photoflash for photography, both professional and consumer. These capacitors are now also used more and more for laser flashlamp discharges. The military is interested in aluminum electrolytics for low-voltage pulsing of diode-pumped laser radars. For the purposes of this article, aluminum electrolytic discharge capacitors are classified into three voltage regimes: 1. High voltage greater than or equal to 350 volts rating. 2. Medium voltage less than 350 volts but greater than or equal to 150 volts rating. 3. Low voltage less than 150 volts rating.
Photoflash applications
Photoflash capacitors used in built-in consumer camera applications are generally in the range of 100 µF 360 V, and may approach several hundreds of microfarads in the separate camera-top units. These small units are often constructed with two porous anodes side-by-side. Typical energy density is 1.5 J/gram or 2 J/cm3. Professional photographers use banks of electrolytic capacitors in portable but portly units weighing 10 kg or so. These contain many thousand of microfarads, usually in switchable, fan-cooled banks. These are generally screw-terminal capacitors whose construction is very similar to normal, filter capacitors. Photoflash capacitors may be used at an average rate of up to eight flashes per minute, depending on the size, energy, and thermal management. Four flashes per minute is more typical. Photoflash operation often causes an adiabatic internal temperature rise of about 0.05 oC (0.09 oF) per flash. This leads to the conclusion that a few hundred flashes is needed to warm the capacitor appreciably. Therefore, for the first half-hour, one could apply 10 flashes per minute sans souci. Typical flash capacitor life is 50,000 to 200,000 flashes. Long-life designs are available to one million or more flashes.
Strobe applications
Strobe capacitors are used at high repetition rates. In the case of low-voltage units, the repetition rate may be very high, high enough for use in party strobe lights and in automotive tachometer lights. High-voltage units generally cannot be operated in full charge-discharge mode beyond about 2 or 3 Hz repetition rate, or rep rate. High-voltage aluminum electrolytic strobe capacitors use a different alumina dielectric structure than their photoflash and filter counterparts. Strobe capacitors use amorphous alumina rather than the usual crystalline alumina. This is accomplished through the forming process when the foil is anodized. Different pre-processing, process temperature and current density, and different electrolyte chemistry is used. The resulting dielectric is unfortunately much thicker than its crystalline counterpart. For this reason, strobe foil has larger tunnels and strobe capacitors suffer in energy density and cost by a factor of about four against their crystalline cousins. But their repetition rates may offer a factor of twenty improvement, and their life duration may approach 1,000 times the number of sustained charge-discharge cycles. When one only needs a fractional discharge, such as discharging from 400 V to 250 V, instead of full-discharge, hybrid capacitor designs can be developed which offer high repetition rate, long life, without the sacrifices in cost and size required by amorphous foil.
In a normal discharge capacitor application, the capacitor is charged slowly, discharged rapidly, and undergoes a certain number of discharge cycles per unit time. The amount of time required for the capacitor to become charged is referred to as the charge time. The amount of time during which the capacitor is discharged is called the discharge time. A charge-discharge cycle is known as a shot. The number of charge-discharge cycles per second is called the repetition rate, expressed in hertz (Hz). When the repetition rate is very small or the capacitor is not fired often, the operating condition is known as single-shot. When a capacitor undergoes intermittent repetition-rated operation, the duty factor is defined as the time on divided by the sum of the time on and the rest period. The life of a capacitor is defined as the expected number of shots before a specified amount of degradation occurs. Usually the limit is an increase in the ESR.
Related articles
Anodizing
Electrochemical capacitors
Further reading
Bibliography
- Many relevant publications are listed in a website by FaradNet.
Listings of electrochemistry books, review chapters, proceedings volumes, and full text of some historical publications are also available in the Electrochemistry Science and Technology Information Resource (ESTIR). (http://knowledge.electrochem.org/estir/)
Return to:
Top
Encyclopedia Home Page
Table of Contents
Author Index
Subject Index
Search
Dictionary
ESTIR Home Page
ECS Home Page
|